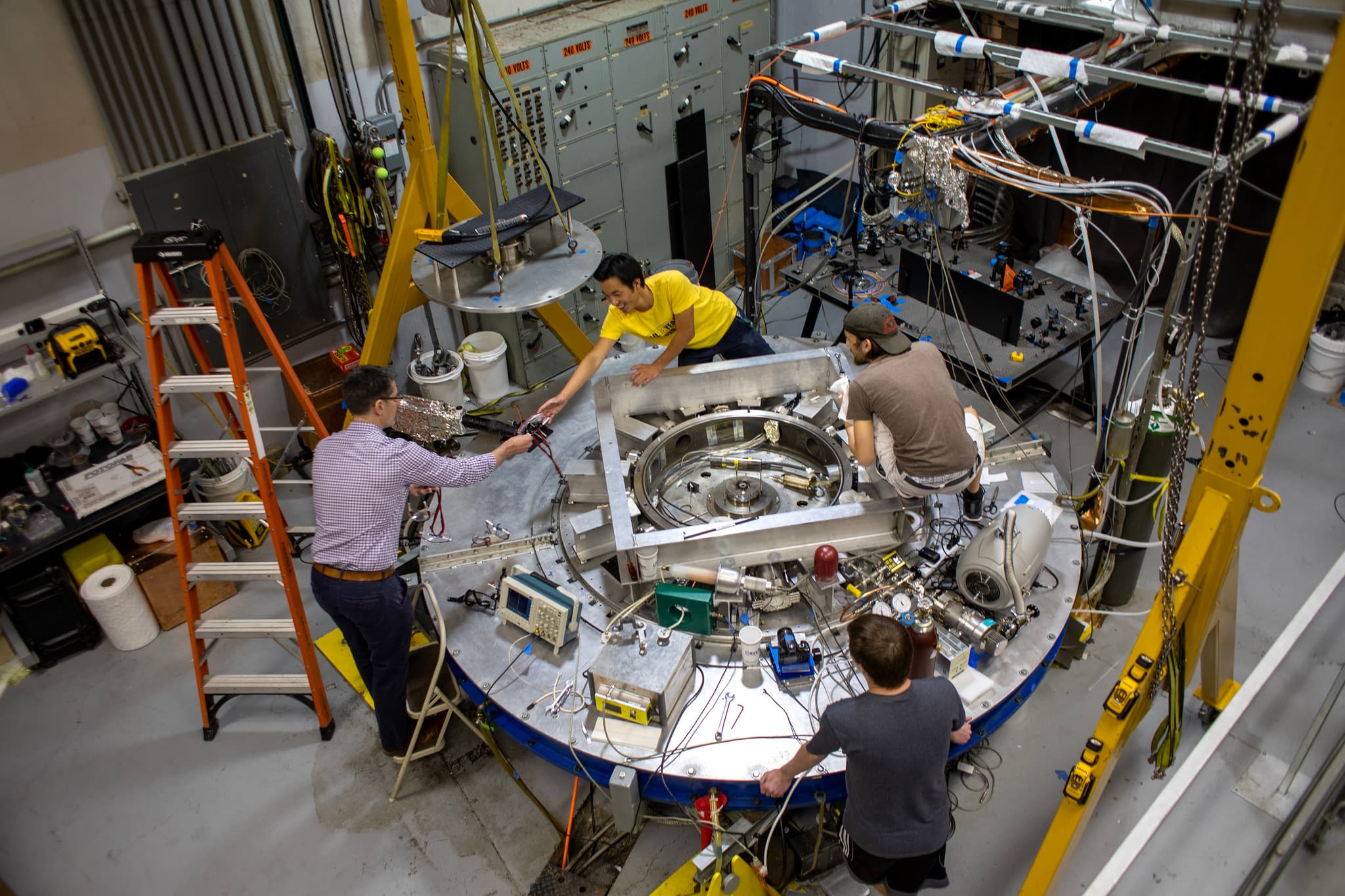
Plasmas & Nuclear Fusion
Plasmas have the potential to purify water, propel spacecraft across the solar system and beyond, heal wounds, ignite nuclear fusion and more.
Our experimental plasma research programs concern topics related to advanced accelerators and lasers, Z-pinches, magnetic and inertial confinement fusion, radiation generation, plasma thrusters, materials processing, low-temperature plasmas, and applications.
We also study basic plasma science questions, investigating why plasmas self-organize, the nature of plasma diffusion through sheaths and magnetic cusps, the nature of the plasma liquid interface, and more.
Our laser capabilities include ZEUS, a three-petawatt system (three petawatts are equal to three quadrillion watts, or a 3 followed by 15 zeros) and the most powerful laser in the United States. Funded by the National Science Foundation, the facility enables both basic and applied research. It will test a leading theory on how the universe operates at a subatomic level, and it could lead to advancements in medicine, materials science, and national security.
Faculty
Labs & Groups
Applied Nuclear Science Group (Prof. Igor Jovanovic) |
Center for High Energy Density Laboratory Astrophysics Research (CHEDAR) (Prof. Carolyn Kuranz) |
Center for Magnetic Acceleration, Compression and Heating (MACH) (Prof. Ryan McBride) |
Computational Plasma Science and Engineering Group (Prof. Mark J. Kushner) |
Gérard Mourou Center for Ultrafast Optical Science (Prof. Karl Krushelnick) |
High Field Science Group (Prof. Alec Thomas) |
Kuranz Astrophysics Team (KRAPHT) (Prof. Carolyn Kuranz) |
Plasma, Beams, and Interface Science Group (Prof. Peng Zhang) |
Plasma Science and Technology Laboratory (Prof. John Foster) |
Plasma, Pulsed Power, and Microwave Laboratory (Prof. Ryan McBride, Prof. Ron Gilgenbach, Prof. Y.Y. Lau) |
Plasma Theory Group (Prof. Scott Baalrud) |
NEWS
GET INVOLVED
We believe that engaging in research as an undergraduate student is a very important part of the NERS experience, and many of our third- and fourth-year undergraduate students are actively involved and have co-authored papers in scientific journals.
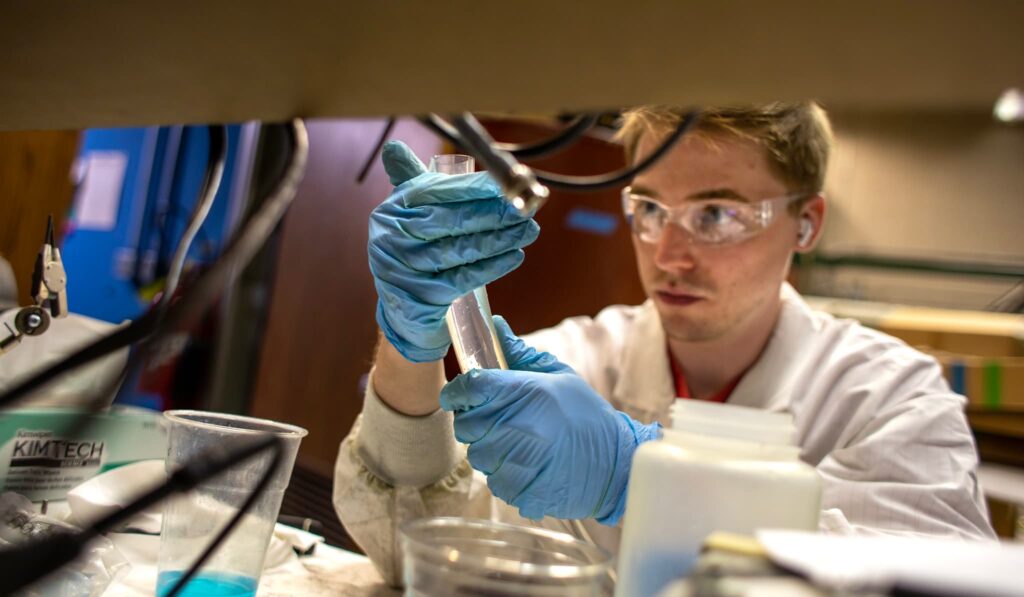